Genetics in the diagnosis and treatment of cardiovascular diseases
Abstract
Cardiovascular diseases (CVDs) remain one of the leading causes of morbidity and mortality worldwide, with genetics being a major risk factor. Genetic cardiovascular disease can occur either because of single variant (Mendelian) or polygenic influences and has been linked to inherited cardiovascular conditions (ICC) such as arrhythmias, cardiomyopathies, dyslipidemias, and aortopathies which are significant factors leading to sudden cardiac death in young adults. Timely screening, diagnosis, and management of ICC can not only provide life-saving treatment to a patient, but also identify at-risk family members. The field of pharmacogenomics (PGx) helped to understand the variable action of medications such as clopidogrel, aspirin, warfarin, and statin according to genotype. Newer technologies such as multi-omics can combine data from multiple sources such as genomics, epigenomics, transcriptomics, proteomics, metabolomics, and microbiome. These advancements can contribute to the development of polygenic prediction scores and precision medicine tailored to individual genotypes. Substantial strides have been made in genetic-based therapeutics, gene editing technologies, and drug delivery systems, which have significantly expanded treatment options for patients with acquired or inherited CVDs. Although variable, the country- and society-specific guidelines on genetic testing for ICC and PGx and treatment are being continuously updated to keep up with ongoing research in the field. Along with appropriate knowledge, other factors including cost and availability of genetic testing play a vital role in the usage by both physicians and patients. With the advent of newer genetic testing for CVDs, a key factor is the availability of genetic counselors (GCs) who are specifically trained in cardiovascular genomics. The current review provides a concise summary of the major influences of genetics in the diagnosis and treatment of CVDs.
Keywords
INTRODUCTION
Cardiovascular disease (CVD) remains the leading cause of mortality worldwide. Nearly one-third of all global deaths were due to CVDs, of which 85% constituted heart attacks and strokes[1]. This trend is seen despite the innovations and improvements in pharmacotherapy such as antiplatelet and cholesterol-lowering agents, likely influenced by underlying variations in genotypes which lead to variable treatment responses[2]. Exploring the underlying genetic mechanisms of CVD has led to developments in the field of precision medicine, wherein tailored therapeutic options can be presented to individual patients for the best possible outcomes[3]. Tailored options have included cytochrome P450 (CYP) 2C19 genetic testing to personalize a P2Y12 inhibitor class of antiplatelets, microRNA (miRNA) biomarkers that diagnose medication-induced cardiotoxicity, and polygenic score to identify increased risk for the development of coronary artery disease (CAD)[4-6]. Although beneficial, concerns remain pertaining to the cost of genetic testing, limited access to genetics healthcare professionals, and a lack of knowledge regarding the interpretation of genetic testing results, which may hinder the utilization of genetics in clinical practice[7-10]. The purpose of this manuscript is to provide a high-level overall review of the current role of genetics in the diagnosis and treatment of CVDs, including the benefits, limitations, and approved testing options and guidelines by society experts.
Advances in genomic testing
There have been several advancements in genomic testing recently, especially for CVDs. These range from targeted sequencing of the genome to multi-omics approaches[4]. Non-coding gene fragments such as microRNAs (miRNAs or miRs) have emerged as potential biomarkers of CVD development and prognosis[11]. These serve as regulators of many genes involved in inflammation and fibrosis, thus controlling CVD evolution. Animal models have shown the downregulation of miR-181a as the mechanism behind decreased myocardial fibrosis seen with sacubitril/valsartan[12]. As biomarkers, the upregulation of some miRNAs has been reported to correlate with heart failure (HF) (miR-122), atrial fibrillation (miR-483-5p), myocardial complication in diabetes
Long and circular RNAs (circ RNAs) are other types of non-coding RNAs that affect gene regulation via epigenetic mechanisms, thereby playing a role in plaque formation and chronic HF[19]. Like miRNAs, several circ RNAs could be used as biomarkers for CAD (hsa_circ_0001879, hsa_circ_0004104, hsa_circ_0005540, hsa_circ_0124644), myocardial infarction (MI) (circRNA_104761), hypertension (hsa-circ-0005870, hsa_circ_0105015 combined with hsa-miR-637), and rheumatic valvular heart disease (hsa_circ_0000437)[20-22]. In the field of cardio-oncology, circFOXO3 and circITCH were shown to affect anthracycline-induced cardiotoxicity[23]. Despite the potential benefits, the clinical application of testing for long non-coding miRNAs and circ RNAs remains limited due to the lack of adequate human studies and trials[24].
Combining data from multiple sources such as genomics, epigenomics, transcriptomics, proteomics, metabolomics, and microbiome, the multi-omics technologies provide a comprehensive diagnostic approach and are the latest upcoming genetic technologies[4]. Multi-omics technologies are being developed and utilized for various CVDs. In a study of healthy subjects, Sánchez-Cabo et al. used blood multi-omics to show the association between subclinical atherosclerosis and epigenetic age acceleration, the latter being defined as the difference between epigenetic age (assessed by the effect of DNA methylation on genome) and chronological age[25]. They reported several inflammatory genes and pathways such as IL1B and IL6 to be responsible for this association. Similar applications of multi-omics in platelets revealed unique phosphopeptides and proteins, helping to better understand platelet activation[26]. Ferrucci et al. performed transcriptomic and proteomic analyses on muscle biopsies in patients with peripheral artery disease and showed alteration of mitochondrial respiratory proteins and glycolytic enzymes, which could be targeted in the future to change disease course[27]. Current atherosclerotic CVD (ASCVD) risk prediction scores are being enhanced with additional input from proteomics and lipidomics such as sphingolipids and fatty acids[28]. In a study of cardiac tissues from patients with hypertrophic cardiomyopathy (HCM),
Single-cell RNA (scRNA) sequencing and transcriptomics provide information from a single cell instead of combined information from multiple tissue cells[36,37]. Currently being extensively tested for use in other fields such as oncology, single-cell sequencing is being explored now for CVDs as well. Single-cell sequencing is being used to understand the pathophysiology of cardiac fibrosis in HF, the role of vascular smooth muscle cells in atherosclerosis, and differences in markers such as ANKRD1 and NPPB according to the site of myocardial ischemia, etc.[36,37]. While most of the work has been done on animal models, replication in human cardiomyocytes is technically challenging due to different cellular structures[37].
Several other new genetic testing modalities are upcoming. Small RNAs derived from transfer RNAs (tRNAs) can function as biomarkers for atherosclerosis, pulmonary hypertension, and MI[38]. Similarly, epigenetic changes in RNA, such as m5C methylation, can lead to CVDs via dysregulated expression[39]. Alteration of apoptosis via the mammalian sterile 20-like kinase 1 (MST1) has been linked with various CVDs such as MI, HF, cardiomyopathy, and aortic vascular diseases[40,41]. Specific galectins such as galactose-specific lectin-3 (galectin-3) have been shown to participate in vascular calcification[42]. These genetic findings would also potentially serve as therapeutic targets for CVDs[43-46].
Advances in genetic-based cardiovascular therapeutics
Advances in genetic-based therapeutics, gene editing technologies, and drug delivery systems have significantly expanded treatment options for patients with acquired or inherited CVDs. Gene therapy presents three new areas of exploration for future application: RNA therapeutics, cardiac regeneration, and cardiac gene editing. RNA therapeutics can now target previously “undruggable” pathways by silencing the expression of proteins involved in pathogenesis or by providing an exogenous message to generate a therapeutic protein[47]. Several RNA therapeutics have proven to be beneficial for treating both rare and highly prevalent CV-related disorders such as atherogenic dyslipidemia[47-49].
In theory, RNA molecules such as antisense oligonucleotides (ASOs), microRNAs (miRNAs), and small interfering RNA (siRNAs) can target any interest gene’s mRNA and non-coding RNAs (ncRNAs). Antisense oligonucleotides (ASOs) selectively bind via complementary base-pairing to mRNA through two mechanisms: occupancy-mediated and occupancy-only. Both binding mechanisms can alter mRNA to modulate protein expression. The occupancy-mediated mechanism of ASOs enhances the downregulation of target transcripts, while the occupancy-only mechanism can control the down-/upregulation of target transcripts. ASOs provide a unique characteristic of steric blocking to allow the regulation of pre-mRNA and mRNA without degrading the RNA itself. Both small interfering RNAs (siRNAs) and microRNA (miRNAs) utilize the RNA-induced silencing complex (RISC) to stop the expression of a targeted protein. siRNAs instigate mRNA degradation, leading to suppression of gene expression at a post-transcriptional level, while miRNAs utilize this pathway to repress translation[47-49].
Preclinical evidence has supported the use of gene therapy, specifically with cytokines, growth factors, and miRNAs, to promote cardiac tissue remodeling for the treatment of conditions such as CAD, PAD, and HF. While multiple animal studies have succeeded in demonstrating angiogenesis, stimulating the regeneration of cardiomyocytes, and reducing oxidative stress and apoptosis, it has not translated into success in clinical trials[50]. However, with advances in gene editing technologies (CRISPR-Cas9, base editing, and prime editing) and direct delivery systems using adeno-associated virus (AAVs), lipid nanoparticles (LNPs), such as liver siRNAs, and virus-like particles (VLPs), the likelihood of success is promising in the near future[50]. There are currently several ongoing clinical trials (ranging from Phase I to III) investigating the use of gene therapy treatment for cardiac regeneration, specifically analyzing gene vehicles and route administration for successful nucleic acid delivery[50].
GENETIC TESTING FOR INHERITED CARDIOVASCULAR DISEASES
Inherited cardiovascular diseases (ICVDs) such as aortopathies, arrhythmias, cardiomyopathies, dyslipidemias, and hypertension are important causes of morbidity and mortality with known pathogenetic mutations [Table 1][1]. Although guidelines have recommended genetic testing for screening ICC, implementation remains low due to a lack of access to genetics healthcare professionals as well as genetic counseling and genetic testing services[51]. Timely screening, diagnosis, and management of inherited CVDs can not only provide life-saving treatment to a patient but also identify at-risk family members. Genetic counseling plays a vital role as a bridge between physicians and patients, helping both to understand genetic testing options and the implications of results[51].
Genetic testing for inherited cardiovascular diseases with definitive, strong, and moderate clinical validity classification
CVD | ICVD and gene-disease classification* | Prevalence [Ref.] |
Aortopathies | Thoracic aortic aneurysms and dissections Definitive: TGFB2, SMAD 3, FBN1. TGFBR1, TGFBR2, ACTA2,MYH11 Strong: MYLK, PRKG1, LOX Moderate: EFEMP2, SMAD2, MFAP5, FOXE3 Ehlers-danlos syndrome Definitive: COL1A1, COL1A2, COL3A1, COL5A1, COL5A2 Loeys-dietz syndrome Definitive: TGFBR1, TGFBR2 Marfan syndrome Definitive: FBN1 | 1.3%-8.9% men; 0.1%-2.2% women [183] 0.002% [52] Unknown 0.005%-0.01% [184] |
Arrhythmias | Long QT syndrome Definitive: CALM1. CALM2, CALM3, SCN5A. KCNH2, KCNQ1 Strong: TRDN Moderate: CACNA1C Short QT syndrome Definitive: KCNH2 Strong: KCNQ1 Moderate: SLC4A3 Catecholaminergic polymorphic ventricular tachycardia Definitive: TRDN, RYR2, CASQ2, TECRL Moderate: CALM1, CALM2, CALM3 Arrhythmogenic right ventricular cardiomyopathy Definitive: CASQ2, RYR2, TECRL, TRDN Moderate: CALM1, CALM2, CALM3, CASQ2 Brugada syndrome Definitive: SCN5A | 0.05% [185,186] 0.05%-0.4% [187,188] 0.01%-0.02% [189] 0.02%-0.05% [190] 0.05% [191] |
Cardio myopathies | Hypertrophic cardiomyopathy Definitive: TNNC1, ACTC1, TPM1, TNNI3, CSRP3, TNNT2, MYH7, MYL3, MYL2, MYBPC3, ALPK3, PRKAG2 Moderate: JPH2, TRIM63, CSRP3, KLHL24 Dilated cardiomyopathy Definitive: BAG3, DES, FLNC, LMNA, MYH7, RBM20, SCN5A, TNNC1, TNNT2, TTN Moderate: ACTC1, JPH2, NEXN, TNNI3, TPM1, VCL Arrhythmogenic right ventricular cardiomyopathy Definitive: DSG2, PKP2 Moderate: DES, PLN | 0.2%-0.5% [192] 0.4% [193] 0.04% [194] |
Congenital heart disease | Congenital heart disease (congenital structural, conductive, myopathic) Definitive: GATA4, SMAD2, NKX2.5, NR2F Moderate: HAND1,HAND2, ETS1 | 0.69%-0.93% [195] |
Dyslipidemias | Familial hypercholesterolemia Definitive: APOB, LDLR, LDLRAP1, PCSK9 Familial hypobetalipoproteinemia Definitive: ANGPTL3, APOB Sitosterolemia Definitive: ABCG5, ABCG8. | 0.32% [196] Unknown 0.0005% [197,198] |
Hypertension | Pulmonary arterial hypertension Definitive: SOX17, BMPR2, KDR, GDF2, TBX4, KCNK3, SMAD9, ATP13A3, CAV1 Moderate: GGCX, TET2, ABCC8 | 0.00106% [199] |
Inherited aortopathies
Inherited aortopathies include Marfan syndrome, Loeys-Dietz syndrome, and Ehlers-Danlos syndrome (EDS). In a retrospective study of the UK National Diagnostic Service, Bowen et al. showed that angiotensin II receptor blocker and beta-blocker therapy can reduce adverse vascular events in Vascular EDS (vEDS), an inherited connective tissue disease which occurs due to COL3A1 genetic variants[52]. Giuliani et al. reported that patients with genetic aortopathies such as Marfan syndrome and Loeys-Dietz syndrome have a higher risk of complications from an aberrant subclavian artery, including aneurysms and dissections[53]. Given the usual poor prognosis associated with ICVDs, there is a huge potential for ongoing and future studies on pharmacogenomics in this field.
Inherited arrhythmia syndromes
Inherited arrhythmia syndromes (IASs) include conditions such as catecholaminergic polymorphic ventricular tachycardia, Brugada syndrome, and long and short QT syndromes, which occur due to genetic variants in genes affecting calcium and ion channels[54]. Clinical genetic testing panels have been created and incorporated into the International Consortium (Clinical Genome Resource)[54]. Even though there exists a significant level of incomplete penetrance among rare mutations in conditions like long QT syndrome and idiopathic atrioventricular conduction disease in young patients, the upcoming therapeutic techniques such as CRISPR/Cas9 technologies may offer a treatment for such disorders by splicing the genetic arrhythmogenic substrates[55-57]. Atrial fibrillation (AF) is the most common cardiac dysrhythmia, with an estimated prevalence from < 0.5% in young adults to > 8% after the age of 80. The heritability of AF is estimated as high as 62%, indicating a strong genetic component[58]. While studies have implicated several pathogenic contributors to AF, there has been no definitive association established[59].
Inherited cardiomyopathies
Inherited cardiomyopathies (ICs) include hypertrophic cardiomyopathy (HCM), dilated cardiomyopathy (DCM), arrhythmogenic right ventricular cardiomyopathy (ARVC), left ventricular noncompaction cardiomyopathy (LVNC), and restrictive cardiomyopathy[60]. Most ICs are single-gene disorders with an autosomal dominant inheritance pattern and a 50% risk of transmission to a child[54]. There is also considerable overlap among the cardiomyopathies[61]. While the association of several genes in relation to HCM, DCM, and ARVC have been established (see Table 1), no genes have been validated in association with restrictive cardiomyopathy. This is despite the fact that numerous pathogenic mutations in almost
Congenital heart disease
Congenital heart diseases (CHD) include ventricular and atrial defects, patent ductus arteriosus, tetralogy of Fallot, pulmonary valve stenosis, and other developmental heart defects that occur in 1% of infants born each year. Like many diseases, CHDs have been associated with environmental and maternal risk factors including smoking, alcohol consumption, age, and obesity, and approximately 20% have been attributed to chromosomal anomalies or genetic defect underpinnings. Point mutations of cardiac transcription factor genes, SNPs, aneuploidy, and chromosomal copy number variants (CNV) are directly associated with CHD pathogenesis[64]. Accordingly, gene-editing technologies such as Zinc Finger Nuclease and CRISPR-Cas9 show the potential to correct genetic mutations that underlie inherited cardiac disorders, which has already been demonstrated in long QT syndrome[65,66]. By precisely targeting and modifying the affected genes, scientists can potentially reverse or mitigate the effects of these genetic abnormalities[67,68].
Several challenges that providers face include identifying the person in a family to begin the genetic testing process, finding the most useful genetic testing panel, and interpreting the genetic testing results[60]. Hence, diagnosing cardiomyopathies and CHD at the earliest stage possible, from prenatal to postmortem diagnosis, has been proposed as it can help in prognostication and identifying other family members at risk[69-72]. While guidelines may recommend genetic testing, significant challenges remain in the operationalization of genetic testing in the clinical care of cardiology patients[73,74]. Another challenge pertains to the prevalence of extracardiac anomalies associated with congenital heart disease and its management. It is estimated that the rate of extracardiac anomalies coupled with CHDs is around 28% and extracardiac structural malformations around 25%[75]. Such cases are more common than isolated cardiac dysfunction and management is becoming increasingly complex. Coordination among different teams and specialists becomes even more imperative, and the role of surgical management in addressing phenotypic malformations is crucial[76].
Dyslipidemias
Genetic causes of dyslipidemia can be monogenic, caused by a rare DNA variant that has a strong impact on phenotype, or polygenic, with multiple common genetic variants. Familial Hypercholesterolemia (FH) is common among the general population, with a prevalence of 1 in 200 to 1 in 250 and remains vastly underdiagnosed and undertreated[77]. Untreated FH causes increased low-density lipoprotein cholesterol (LDL-C) levels, leading to an increased risk of premature coronary heart disease, which can manifest as myocardial infarctions and sudden cardiac death[77]. Individuals with untreated FH have a 20-fold increase in risk of premature CHD and a 13-fold increase in CHD mortality as compared to the general population[78]. FH is associated with mutations in four proteins: low-density lipoprotein receptor (LDLR), low-density lipoprotein receptor adaptor protein (LDLRAP1), apolipoprotein B (APOB), and proprotein convertase subtilin/kexin 9 (PCSK9)[78].
One of the most notable targeted drug treatments for LDL lowering and atherosclerotic CV disease (ASCVD) risk reductions is PCSK9 inhibitors. PCSK9 is an enzyme that marks LDL receptors for degradation, prevents LDL-C from being broken down intracellularly, and results in elevated levels of circulating LDL-C[79]. Gain of function mutations in the PCSK9 gene increases the activity of the PCSK9 enzyme; thus, treatment in the form of PCSK9 inhibitors, such as monoclonal antibodies, has been indicated in patients with heterozygous FH in which a pathogenic mutation in the PCSK9 gene has been identified. Currently, there are three approved PCSK9 inhibitors that have demonstrated a substantial reduction in LDL levels and reduced major adverse cardiovascular events (MACE) when used in conjunction with statin therapy. Alirocumab (Praluent; Regeneron/Sanofi) and evolocumab (Repatha; Amgen) are fully humanized monoclonal antibodies. Inclisiran (Leqvio; Novartis) is a small interfering mRNA that inhibits the intracellular synthesis of PCSK9. Mipomersen is an antisense oligonucleotide inhibitor that causes selective degradation of the apoB-100 mRNA and inhibition of protein translation, leading to substantial reductions in LDL-C and other lipoprotein levels[72]. In heterozygous FH patients with CHD, LDL-C levels were reduced by 28% when treated with mipomersen[80].
Angiopoietin-like 3 (ANGPTL3) has newly emerged as a target for the treatment of elevated levels of circulating LDL-C in individuals with homozygous familial hypercholesterolemia (HoFH). HoFH is caused by DNA variants in LDLR, and other genes associated with LDLR function, including APOB, PCSK9, and LDLRAP1[81]. Individuals with the rare genetic disorder display severely elevated LDL-C levels due to minimal or no residual LDLR (LDL receptor) function. LDLR-dependent cholesterol-lowering medications such as PSCK9 inhibitors, statins, ezetimibe, and bempedoic acid have minimal effect in lowering LDL-C in individuals with HoFH[82]. Inhibition of ANGPTL3 reduces LDL-C independently of the LDLR potentially by controlling VLDL-C catabolism upstream of LDL-C. Presently, there is one approved ANGPLT3 inhibitor, evinacumab (Evkeeza; Regeneron), a fully humanized monoclonal antibody[81].
Increased serum Lipoprotein (a) (Lp(a)) concentration is an important inherited risk factor for ASCVD and aortic stenosis progression and is independent of the serum LDL-C. Several studies investigating antisense oligonucleotides (ASO) pelcarsen (Ionis Pharmaceuticals/Novartis) and LY3819469, as well as small interfering RNAs (siRNAs) olpasiran (Amgen) and SLN360, have shown to be highly effective in lowering the serum Lp(a) concentration with a good safety profile[83,84]. Upcoming Phase III trial results will determine if these new RNA-based therapies will be a major player in the wide spectrum of lipid-lowering medication. Other genetic forms of FH where expression of some functional LDL receptors can be unregulated are included in Table 1. Although the genetics of FH are well known, the integration of genetic testing into practice has been constrained due to limited knowledge of FH by providers, lack of consensus among FH diagnostic criteria, and time constraints in clinical encounters[85].
CARDIOVASCULAR THERAPY PHARMACOGENOMICS
Genetic variants have been identified as contributors to antiplatelet drug response and other cardiovascular medications [Table 2]. Sinai Center for Thrombosis Research (SCTR), in collaboration with the University of Maryland School of Medicine (UM), was one of the first to test the role of cytochrome P450 (CYP) 2C19*2 variant in the pharmacodynamic and clinical efficacy of clopidogrel therapy[86,87]. In a genome-wide study, healthy participants treated with clopidogrel for 7 days were evaluated for genetic associations with platelet aggregation in the Pharmacogenomics of Antiplatelet Intervention (PAPI) Study[86]. In response to clopidogrel, the presence of CYP2C19*2 was associated with reduced inhibition of ADP-stimulated platelet aggregation. In the initial validation study that evaluated patients who underwent percutaneous coronary intervention (PCI), carriers of the CYP2C19*2 alleles were found to have a significant increase in cardiovascular events at 1 year as compared to non-carriers (20.9% vs. 10.0%). This landmark study was among the first to open the gates to precision medicine, leading to the consideration of alternative antiplatelet therapy (not containing clopidogrel) in patients carrying the loss-of-function CYP2C19*2 genotype.
Cardiovascular medications, single nucleotide polymorphisms and drug response with guideline-based recommendations
CV medication | Gene (SNP ID) | Drug response and guideline-based recommendations |
Clopidogrel | CYP2C19*2 (rs4244285), *3 (rs4986893) CYP2C19*17 (rs12248560) | Response: reduced effectiveness in CYP2C19 LOF carriers in homozygote (poor metabolizers) and heterozygote (intermediate) LOF carriers and increased or normal effectiveness in GOF carriers Recommendations: alternative P2Y12 inhibitors (Ticagrelor or Prasugrel) in poor/ intermediate metabolizers if clinically indicated |
Warfarin | VKORC1 (rs9923231) LOF CYP2C9*2 (rs1799853) LOF CYP2C9*3 (rs1057910) Other: CYP4F2 (rs2108622), CYP3A4 (rs2242480), CYP4F2 (rs2108622) | Response: increased effectiveness (VKORC1 A/G or A/A), Reduced effectiveness in CYP2C9 poor metabolism (e.g., CYP2C9*3/*3, *2/*3, *3/*3). Other gene polymorphisms under investigation or weak evidence Recommendation: consider an alternative agent (DOAC) in individuals with VKORC1 A/G or A/A genotype or CYP2C9 poor metabolizers |
Statin therapy | Statin Induced Myopathy SLCO1B1 (rs4149056, rs4263657) | Response: common variants of the rs4149056 C allele are strongly associated with an increased risk of statin-induced cardiomyopathy. Increased risk for rhabdomyolysis with statin use (rs4263657) Recommendation: consider a tailored statin dose in individuals with the C allele. Advised to avoid statins (rs4263657) |
In the ONSET/OFFSET and RESPOND genotype studies, our group performed the first correlation study between the CYP2C19 genotype and the effect of clopidogrel vs ticagrelor in patients with CAD[88]. Irrespective of the CYP2C19 carrier status, ticagrelor therapy was found to significantly reduce platelet reactivity compared with clopidogrel (P < 0.01). Additionally, the presence of loss-of-function carriage of the CYP2C19 genotype did not affect the antiplatelet action of ticagrelor but was associated with higher platelet reactivity in those on clopidogrel. A subsequent meta-analysis of 9 studies with 9,685 CAD patients treated with clopidogrel similarly reported a significant increase in major adverse cardiovascular events (MACE) and stent thrombosis among carriers of reduced-function CYP2C19 alleles as compared to non-carriers[89]. Such genotypic studies were valuable in understanding experimental antiplatelet therapies as well[90].
Going further, our group evaluated the effect of CYP2C19 in patients on maintenance dual antiplatelet therapy (DAPT) with aspirin and clopidogrel[91]. While no difference was found in platelet reactivity between carriers and non-carriers of the *2 allele in patients on aspirin alone, *2 carriage was associated with higher platelet aggregation in patients on DAPT with clopidogrel. Similar findings were shown in a subset of East Asian patients with MI who inherently had a higher prevalence of CYP2C19 loss-of-function allele carriage[92]. Following this, the guidelines for antiplatelet therapy were modified to incorporate genotypic testing in selecting high-risk patients undergoing PCI, where alternate antiplatelet agents may be selected based on the results[93-96].
In addition to the CYP2C19*2 allele, effects of other loss-of-function alleles such as *3 were evident in the ACCEL-DOUBLE-2N3 study conducted in Korea by Jeong et al.[97]. East Asian patients who underwent PCI and were treated with clopidogrel (standard dose 75 mg or high dose 150 mg) demonstrated increased platelet aggregation with *3 allele carriage as compared with *2 allele. While conventional CYP2C19 genetic testing is time-consuming and labor-intensive, our group was among the first to test and report a high accuracy of a novel point-of-care testing method that could analyze 11 CYP2C19 variants within 3 h[98]. Similar to ticagrelor as above, we also showed no effect of CYP2C19 LoF carriage on platelet reactivity in those treated with prasugrel[99]. Although trials such as TRILOGY ACS failed to show any significant difference in cardiovascular outcomes between prasugrel and clopidogrel based on genetically predicted CYP2C19 metabolizer status in medically treated patients with acute coronary syndrome (ACS), genetic testing held importance for patients with high-risk characteristics[100-102]. This was later confirmed by the genome-wide association study (GWAS) in patients on clopidogrel, which showed a significant association of CYP2C19*2 with platelet reactivity, with the subsequent development of pharmacogenomic polygenic response score showing predictive value for cardiovascular outcomes[103,104]. The work in pharmacogenomics of antiplatelet therapies has recently been conducted in other countries as well, along with studies showing their cost effectiveness as compared with the standard of care[105-111].
Like clopidogrel, resistance to aspirin has been associated with genetic variants. For example, resistance has been linked to variants in the genes encoding GPIIIa and COX[4]. A recent systematic review of 21 studies with 10,873 patients reported 3,014 to be aspirin-resistant[112]. This resistance was attributed to polymorphisms of various genes such as P2RY1, MDR1, PLA2G7, HO1, TBXA2R, ALOX12, ALOX5AP, and PON1. Furthermore, a variant in endothelial aggregation receptor-1 (PEAR1) has been linked to residual platelet aggregation following aspirin treatment[113]. However, in a study of 13,547 healthy older individuals without a history of atherothrombotic CVD, Lewis et al. found no effect of PEAR1 genotype and aspirin use on cardiovascular and bleeding events[114]. A recent healthy volunteer study evaluated 62 pro-thrombotic gene transcripts included in the Aspirin Response Signature (ARS) score and reported that a higher score is associated with increased platelet inhibition and bleeding risk. These findings suggest that ARS scores may be used to guide aspirin treatment[115]. As the evidence for any specific gene involved in aspirin resistance remains low and heterogeneous, future studies will play an important role in precision medicine with aspirin[4].
Anticoagulant medications are used for various indications, including prevention of stroke in the setting of atrial fibrillation, to treat venous thromboembolism, etc.[4]. Warfarin was previously the most common anticoagulant used and was replaced later by direct oral anticoagulants (DOACs). Although genetic studies revealed a variation in the effectiveness of warfarin due to genotypic variants in CYP2C9 (warfarin metabolism), VKORC1 (target enzyme), and CYP4F2 (vitamin K metabolism), the subsequent clinical trials reported conflicting results on the role of implementing genotype-guided algorithms for warfarin[4,116,117]. This was likely due to the geographical, racial, and dosing heterogeneity in patient groups[4]. Similar studies have also been recently conducted to assess the role of pharmacogenomics for DOACs, which could be used to predict the risk of bleeding in the future[118,119].
For statins, the SLCO1B1 gene variant is the most studied and has been shown to be associated with statin-induced myopathy due to decreased clearance by the liver[4,120,121]. ABCG2 encodes an efflux transporter (BCRP) that modulates the absorption and disposition of rosuvastatin with carriers of A allele experiencing a greater reduction in LDLc. However, many genetic variants, such as rs2231142 in ABCG2, have wide variation in prevalence in different patient populations, as evidenced by recent studies[122,123]. Another genotype (LILRB5 Asp247Gly) was found to cause an increase in creatine kinase (CK), which could possibly contribute to precision therapy in the future[124]. The potential of pharmacogenomics in prescribing statins is vast, given that almost 40 variants of 23 genes were found to be linked with dyslipidemia in a recent genomic study[125].
Glucagon-like peptide-1 receptor agonists (GLP-1 agonists) are one of the latest therapeutic options that have been shown to reduce adverse cardiovascular outcomes in addition to being used for diabetes[126]. A genomic study recently reported a greater reduction in HbA1C with GLP-1 agonists in the presence of ARRB1 genetic variants, which would be important to assess for cardiovascular risk reduction in the future[126]. Such advances in pharmacogenomics coupled with other medications with an indirect effect on CVDs show a promising future for precision medicine[127-130].
The development of clinical trials that include PGx information may help to improve our understanding of the mechanism of action of drugs used for the treatment. Furthermore, there is growing evidence, primarily from oncology trials, that the incorporation of PGx within study design leads to lower drug development costs and increased safety and efficacy[131]. A growing number of clinical trials with PGx information are appearing in the field of cardiovascular medicine. As an example, Tardif et al. performed a retrospective GWAS study of the overall neutral placebo-controlled dal-Outcomes trial and found that the effect of the cholesteryl ester transfer protein (CETP) modulator dalcetrapib on the composite of cardiovascular death, myocardial infarction or stroke was influenced by a polymorphism in the adenylate cyclase type 9 (ADCY9) gene[132]. Although patients with the AA genotype at position rs1967309 experienced fewer cardiovascular events with dalcetrapib, those with the GG genotype had an increased rate and the heterozygous AG genotype exhibited no difference from placebo. The role of dalcetrapib in reducing the occurrence of MI in patients with recent ACS and AA genotype at variant rs1967309 (ADCY9 gene) is under investigation in the dal-GenE trial [Supplementary Table 1]. Additionally, PGx is currently utilized to evaluate pharmacogenetic interactions of RNAi therapeutics in development, including PCSK-9 and Lp(a) inhibitors.
IMPLEMENTATION INTO MEDICAL PRACTICE
Various country- or society-specific guidelines exist on genetic testing for CVDs and pharmacogenomics. The American Heart Association (AHA) and American College of Cardiology (ACC) guidelines recommend genetic testing for patients with suspected or confirmed diagnosis of inherited CVD along with cascade testing for family members of patients with HCM, other forms of inherited cardiomyopathies, arrhythmias, familial hypercholesterolemia, heritable thoracic aortic aneurysm, or dissection, and left ventricular noncompaction with shared decision making, pre and post genetic counseling[133,134]. For pediatric patients, special consideration was advised for predictive genetic testing due to the incomplete penetrance of most CVDs, thereby providing a patient with a genetic diagnosis that may not manifest clinically in their lifetime[135]. Additionally, while the Genetic Information Nondiscrimination Act protects an individual’s genetic information from health insurance discrimination and employer discrimination, it does not provide protection for life insurance and long-term disability insurance[134]. Given the implications of positive genetic results for asymptomatic individuals such as the pediatric population, the timing of such testing in the pediatric population was advised to be delayed till the patient reaches decision-making age, unless a disease-modifying treatment is available for the genetic condition being considered.
In 2022, the ACC/AHA released detailed guidelines for aortic diseases, specifically elaborating on the genetic testing, follow up, and management of hereditary thoracic aortic diseases (TAD)[136]. Key risk factors for familial TAD include the presence of syndromes such as Marfan syndrome, occurrence of TAD
Like inherited CVDs, various committees have provided guidelines for pharmacogenomic testing. These include the Clinical Pharmacogenetics Implementation Consortium (CPIC), the Dutch Pharmacogenetics Working Group (DPWG), the Canadian Pharmacogenomics Network for Drug Safety (CPNDS), etc.[142]. Based on the committee recommendations and the tables by the Food and Drug Administration (FDA) on pharmacogenetic biomarkers/associations, the American College of Medical Genetics and Genomics (ACMG) has provided guidance to the laboratories conducting the genetic testing[143,144].
As opposed to inherited CVDs, routine pharmacogenomic testing is not advised. Although CPIC is recommending genetic testing to optimize medication use, their adoption in the clinic remains uncommon. If available, the CPIC guidelines advise therapeutic warfarin dosing based on the CYP2C9 and VKORC1 genotypes to maintain the target international normalized ratio (INR)[145]. For clopidogrel, only selective testing of the CYP2C19 genotype was advised in high-risk patients undergoing PCI at the discretion of the treating physician[146,147]. Regarding statin-associated musculoskeletal symptoms, the CPIC recommended dosing based on the SLCO1B1, ABCG2, and CYP2C9 genotypes[148]. While Medicare provides coverage for pharmacogenomic testing based on the FDA and CPIC guidelines, the inconsistencies between the genetic lists of these organizations need better corroboration[149,150].
Genetic testing for affected patients can include single site testing once a specific genetic variant is identified within a family, phenotype-specific panels (i.e., Familial Hypercholesterolemia panel, Brugada syndrome panel, etc.), or expanded panels that include genes that are associated with multiple cardiovascular conditions. The utility of genetic testing is vast, including reproductive decision-making, surgical or pharmacological treatment, and management of cardiological and extra-cardiological symptoms.
The cost and accessibility of genetic testing have improved over the last 15 years due to technological advancements, an increase in commercial companies and university-based programs, and the incorporation of point-of-care (POC) genetic testing [Supplementary Table 2] However, there are important limitations to be considered regarding genetic testing for CVDs. First, obtaining insurance coverage for expanded testing can be a distinct barrier for some patients. In Dellefave-Castillo’s (2022) study, comprehensive genetic testing was provided through a sponsored program at no cost for the patients[151]. However, in mainstream clinical practice, genetic testing is traditionally billed through insurance by the laboratory, with any remaining cost billed to the patient directly. Large deductibles and lack of insurance coverage for broader panels could lead to large out-of-pocket costs for patients. While many labs offer financial assistance plans and ways to bypass insurance, the prices patients must pay can still be prohibitive for many families. There are also many programs for patients who need to have genetic testing done, so that they can even do genetic testing free of charge in certain cases.
Second, as the number of genes tested increases, so does the possibility of receiving uncertain results such as Variants of Uncertain Significance (VUSs)[152]. VUSs are mutations with limited or conflicting evidence to support whether they are pathogenic or benign[153]. This ambiguity can lead to confusion among providers and patients alike[154]. While guidelines recommend that VUSs should not be used to guide medical decision-making or surveillance of at-risk family members, these types of results may lead to more questions than answers[155]. Over time, many of these variants are reclassified to either benign or pathogenic, but the timeline of reclassification spanning from months to years may lead to higher levels of concern, ambiguity in clinical and reproductive decision-making, and inappropriate discharge from follow up[152,156]. Providers should take special care in explaining the possibility of a VUS in pre- and post-test counseling.
Third, choosing a test that includes genes conferring risk for cardiovascular phenotypes beyond the patient’s clinical diagnosis increases the risk for secondary findings. Secondary findings (i.e., incidental findings) are results unrelated to the diagnostic question or original purpose of the genetic test[156]. Whether one would classify a new molecular diagnosis of cardiomyopathy in a patient clinically diagnosed with an arrhythmia as a secondary finding is up to the frame of reference of whether the purpose of the test is to elucidate genetic changes that may contribute to their heart problems or is it to confirm/deny whether a mutation exists in genes only related to their cardiomyopathy. Our understanding of cardiovascular genotype-phenotype associations is evolving, and an unexpected result may be phenotypic expansion. Regardless, patients may experience surprise or anxiety when receiving a diagnosis that is different from their reason for referral. Similarly, providers may not feel equipped to disclose these results. For example, an electrophysiologist may not feel as prepared as a cardiomyopathy specialist to discuss the implications of an unexpected genetic test result conferring a risk for cardiomyopathy. Providers and patients should both be informed that new cardiovascular diagnoses may be revealed through comprehensive cardiovascular genetic testing. Pre-test counseling should include the benefits and risks of genetic testing so patients can make informed decisions about their health.
With respect to pharmacogenomics, there are chances of errors that may lead to false under or overdosing of the medication[145]. Unlike established forms of CVDs, the insurance coverage for genetic testing remains incoherent[139-141,149,150]. Additionally, medications such as warfarin have few indications left due to the era of DOACs, and INR monitoring is still needed after genetic testing is done[145]. Lastly, the clinicians, especially primary care physicians, may not be up to date regarding the indications and adequate referral for genetic testing, thereby further limiting the awareness among patients.
Treatment and outcomes
The utility of genetic testing and appropriate counseling is predicated on improving patient understanding of their disease and psychological and emotional stress related to their diagnosis and informing treatment plans to increase the likelihood of positive outcomes. Implementing genetic testing in the clinic has already been seen in other fields. McKnight et al. utilized genetic testing to enhance epilepsy treatment, which included adding or beginning a new medication, referring to a specialist, or stopping a current medication. This was coupled with 75% of patients in the study citing positive outcomes and 65% reporting a reduction in seizure activity[157]. Positive outcomes and/or a greater understanding of disease associated with genetic testing have also been seen in the context of primary and preventative care[158,159]. Improved positive outcomes have been demonstrated pertaining to cardiovascular disease, namely reduced risk of MACE and major and minor bleeding during P2Y12 de-escalation[160], significantly reduced LDL-C level in patients with familial hypercholesterolemia without CHD[161], and event-free survival in patients with dilated cardiomyopathy[162]. Notably, gene therapy of mice with ARVC harboring a pathogenic mutation in the PKP2 gene has been shown to restore PKP2 protein levels, inhibit pathogenesis, and prolong survival[163,164].
Genetic counseling
With the advent of newer genetic testing for CVDs, a key factor is the availability of genetic counselors (GCs) who are trained specifically in cardiovascular genomics[165]. Cardiovascular GCs are vital in constructing a thorough and complete three-generation family history (pedigree), determining which genetic testing is most appropriate given an individual’s personal and/or family history, discussing the benefits, limitations, and implications of genetic testing, providing psychosocial support, and coordinating proband (first-person in the family to undergo genetic testing) and cascade genetic testing (testing family members after a disease-causing mutation has been identified in the family)[165]. Additionally, given the nature of cardiovascular genetic testing, GCs also collaborate with regional and state medical examiner officers to coordinate postmortem genetic testing in order to identify the cause of death as well as determine if relatives are at risk for genetic disease and/or sudden death. GCs are well positioned to bridge the silos of the healthcare system and promote team science approaches and interdisciplinary collaboration. While there is not a one-size-fits-all model for the integration of cardiovascular GCs in a clinical practice setting, it is crucial that genetic counseling occurs simultaneously with genetic testing in order to promote patient-centered care and informed decision making. Although effective collaboration and communication are seen in the various practice models used by genetic counselors at different clinical practices, further development into a comprehensive and cohesive model may lead to improved outcomes[166]. Such models for counseling of inherited CVDs are being rapidly updated to keep pace with the evolving genetic testing[167-169].
Polygenic risk scores
Polygenic risk scores (PRSs) are a weighted average of the effects of all genetic variations across the genome contributing to a particular disease and condensed into a single score. The American Heart Association has outlined three categories to consider for implantation into clinical use: efficacy, harm, and logistics[170]. Studies have shown that utilizing genetic scoring can increase the accuracy of predicting risk[171], identifying those who can benefit the most from statin use[172], and estimating lifetime risk[173]. However, given the bias in the genetic databases that are used to construct these scores[174], more research and increased diversity of the dataset would greatly benefit its implementation.
ETHICAL, LEGAL, AND PSYCHOSOCIAL IMPLICATIONS
Given the sensitivity and personal nature of genetic testing, patients may be reluctant and concerned about generating such information. While previous documents address ethical concerns related to research involving human subjects, such as the Nuremberg Code and the Declaration of Helsinki[175,176], they serve as a principal backdrop to build new parameters related to genetic testing. Fear of discrimination by employers and insurance companies, psychological impact of learning about genetic status, and decision-making have been shown as patient concerns[177,178]. Further, premature commercialization and conflict of interest have already been demonstrated[179].
FUTURE PERSPECTIVES
Genetic testing to diagnose CVDs and to guide pharmacotherapies, particularly for precision medicine, has a big potential to modify morbidity and mortality rates in these subsets of patients. The upcoming gene therapies, including targeted siRNAs, can contribute to a significant reduction in adverse CV outcomes in vulnerable populations. Additionally, novel therapies aimed at the sarcomere have shown promise in treating those with various cardiomyopathies[61]. Conspicuously, there is a dearth of information pertaining to pathogenic mutations in genes related to thrombosis and atherosclerosis. Characterizing and validating such genes would provide great insight into cardiovascular pathogenesis. Further, continuing to develop the use of genome editing with CRISP-Cas9 and RNA editing with Cas7-11 holds great potential[180,181]. However, as previous research has shown, there is a significant gap between available evidence and implementation into practice[182]. At the center of this all is the requirement for collaboration among physicians and the wider availability of genetic counselors.
DECLARATIONS
Acknowledgments
We would like to extend my heartfelt appreciation to Toni Tan for her invaluable contributions to the collection of references, technical editing, and proofreading.
Authors’ contributions
Made substantial contributions to the conception and design of the study, prepared the initial draft, and provided edits to the paper: Singh S, Bliden K, Shanoada R, Kalia I, Zimmerman A, Stude T, Gurbel P
Performed editing and critically reviewed the manuscript for important intellectual content: Tantry U, Babu AD, Raghavakurup L, Sidorski D
Availability of data and materials
Not applicable.
Financial support and sponsorship
None.
Conflicts of interest
All authors declared that there are no conflicts of interest.
Ethical approval and consent to participate
Not applicable.
Consent for publication
Not applicable.
Copyright
© The Author(s) 2024.
Supplementary Materials
REFERENCES
1. World Health Organization. Cardiovascular diseases (CVDs) Fact sheet. Available from: https://www.who.int/news-room/fact-sheets/detail/cardiovascular-diseases-(cvds) [Last accessed on 12 Apr 2024].
2. Castrichini M, Luzum JA, Pereira N. Pharmacogenetics of antiplatelet therapy. Annu Rev Pharmacol Toxicol 2023;63:211-29.
3. Wang RS, Maron BA, Loscalzo J. Multiomics network medicine approaches to precision medicine and therapeutics in cardiovascular diseases. Arterioscler Thromb Vasc Biol 2023;43:493-503.
4. Ross S, Krebs K, Paré G, Milani L. Pharmacogenomics in stroke and cardiovascular disease: state of the art. Stroke 2023;54:270-8.
5. Kuang Z, Wu J, Tan Y, Zhu G, Li J, Wu M. MicroRNA in the diagnosis and treatment of doxorubicin-induced cardiotoxicity. Biomolecules 2023;13:568.
6. Christiansen MK, Nyegaard M, Jensen HK. Polygenic risk scores in coronary artery disease. Curr Opin Cardiol 2023;38:39-46.
7. Sethi Y, Patel N, Kaka N, et al. Precision medicine and the future of cardiovascular diseases: a clinically oriented comprehensive review. J Clin Med 2023;12:1799.
8. Catchpool M, Ramchand J, Martyn M, et al. A cost-effectiveness model of genetic testing and periodical clinical screening for the evaluation of families with dilated cardiomyopathy. Genet Med 2019;21:2815-22.
9. Kasztura M, Richard A, Bempong NE, Loncar D, Flahault A. Cost-effectiveness of precision medicine: a scoping review. Int J Public Health 2019;64:1261-71.
10. Kiflen M, Le A, Mao S, et al. Cost-effectiveness of polygenic risk scores to guide statin therapy for cardiovascular disease prevention. Circ Genom Precis Med 2022;15:e003423.
11. Wronska A. The role of microRNA in the development, diagnosis, and treatment of cardiovascular disease: recent developments. J Pharmacol Exp Ther 2023;384:123-32.
12. Vaskova E, Ikeda G, Tada Y, Wahlquist C, Mercola M, Yang PC. Sacubitril/Valsartan improves cardiac function and decreases myocardial fibrosis via downregulation of exosomal miR-181a in a rodent chronic myocardial infarction model. J Am Heart Assoc 2020;9:e015640.
13. Stojkovic S, Koller L, Sulzgruber P, et al. Liver-specific microRNA-122 as prognostic biomarker in patients with chronic systolic heart failure. Int J Cardiol 2020;303:80-5.
14. Wang H, Chen Y, Tao T, et al. Identification of microRNA biomarkers in serum of patients at different stages of atrial fibrillation. Heart Lung 2020;49:902-8.
15. Bejleri J, Jirström E, Donovan P, Williams DJ, Pfeiffer S. Diagnostic and prognostic circulating microrna in acute stroke: a systematic and bioinformatic analysis of current evidence. J Stroke 2021;23:162-82.
16. Pérez-Cremades D, Chen J, Assa C, Feinberg MW. MicroRNA-mediated control of myocardial infarction in diabetes. Trends Cardiovasc Med 2023;33:195-201.
17. Bhatti JS, Khullar N, Vijayvergiya R, Navik U, Bhatti GK, Reddy PH. Mitochondrial miRNA as epigenomic signatures: visualizing aging-associated heart diseases through a new lens. Ageing Res Rev 2023;86:101882.
18. Guo YT, Xiao YC, Xu YL, et al. The effects of microRNAs in the development of heart failure. Curr Cardiol Rep 2023;25:747-59.
19. Samra M, Srivastava K. Non-coding RNA and their potential role in cardiovascular diseases. Gene 2023;851:147011.
20. Long Q, Lv B, Jiang S, Lin J. The landscape of circular RNAs in cardiovascular diseases. Int J Mol Sci 2023;24:4571.
21. Joaquim VHA, Pereira NP, Fernandes T, Oliveira EM. Circular RNAs as a diagnostic and therapeutic target in cardiovascular diseases. Int J Mol Sci 2023;24:2125.
22. Wang K, Gao XQ, Wang T, Zhou LY. The function and therapeutic potential of circular RNA in cardiovascular diseases. Cardiovasc Drugs Ther 2023;37:181-98.
23. Neufeldt D, Cushman S, Bär C, Thum T. Circular RNAs at the intersection of cancer and heart disease: potential therapeutic targets in cardio-oncology. Cardiovasc Res 2023;119:1495-508.
24. Singh DD, Kim Y, Choi SA, Han I, Yadav DK. Clinical significance of microRNAs, long non-coding rnas, and circRNAs in cardiovascular diseases. Cells 2023;12:1629.
25. Sánchez-Cabo F, Fuster V, Silla-Castro JC, et al. Subclinical atherosclerosis and accelerated epigenetic age mediated by inflammation: a multi-omics study. Eur Heart J 2023;44:2698-709.
26. Solari FA, Krahn D, Swieringa F, et al. Multi-omics approaches to study platelet mechanisms. Curr Opin Chem Biol 2023;73:102253.
27. Ferrucci L, Candia J, Ubaida-Mohien C, et al. Transcriptomic and proteomic of gastrocnemius muscle in peripheral artery disease. Circ Res 2023;132:1428-43.
28. Nurmohamed NS, Kraaijenhof JM, Mayr M, et al. Proteomics and lipidomics in atherosclerotic cardiovascular disease risk prediction. Eur Heart J 2023;44:1594-607.
29. Garmany R, Bos JM, Tester DJ, et al. Multi-omic architecture of obstructive hypertrophic cardiomyopathy. Circ Genom Precis Med 2023;16:e003756.
30. Moore J, Ewoldt J, Venturini G, et al. Multi-omics profiling of hypertrophic cardiomyopathy reveals altered mechanisms in mitochondrial dynamics and excitation-contraction coupling. Int J Mol Sci 2023;24:4724.
31. Rega S, Farina F, Bouhuis S, et al. Multi-omics in thoracic aortic aneurysm: the complex road to the simplification. Cell Biosci 2023;13:131.
32. Hasman M, Mayr M, Theofilatos K. Uncovering protein networks in cardiovascular proteomics. Mol Cell Proteom 2023;22:100607.
33. Li Z, Brittan M, Mills NL. A multimodal omics framework to empower target discovery for cardiovascular regeneration. Cardiovasc Drugs Ther 2024;38:223-36.
34. Adua E. Decoding the mechanism of hypertension through multiomics profiling. J Hum Hypertens 2023;37:253-64.
35. Maiorino E, Loscalzo J. Phenomics and robust multiomics data for cardiovascular disease subtyping. Arterioscler Thromb Vasc Biol 2023;43:1111-23.
36. Safabakhsh S, Ma WF, Miller CL, Laksman Z. Cardiovascular utility of single cell RNA-Seq. Curr Opin Cardiol 2023;38:193-200.
37. Miranda AMA, Janbandhu V, Maatz H, et al. Single-cell transcriptomics for the assessment of cardiac disease. Nat Rev Cardiol 2023;20:289-308.
38. Wang S, Luo Z, Yuan L, et al. tRNA-derived small rnas: novel insights into the pathogenesis and treatment of cardiovascular diseases. J Cardiovasc Transl Res 2023;16:300-9.
39. Balachander K, Priyadharsini JV, Roy A, Paramasivam A. Emerging role of RNA m5C modification in cardiovascular diseases. J Cardiovasc Transl Res 2023;16:598-605.
40. Yin Y, Tan M, Han L, et al. The hippo kinases MST1/2 in cardiovascular and metabolic diseases: a promising therapeutic target option for pharmacotherapy. Acta Pharm Sin B 2023;13:1956-75.
41. Shao Y, Wang Y, Sun L, Zhou S, Xu J, Xing D. MST1: A future novel target for cardiac diseases. Int J Biol Macromol 2023;239:124296.
42. Cai Y, Sun Z, Shao C, Wang Z, Li L. Role of galectin-3 in vascular calcification. Glycoconj J 2023;40:149-58.
43. Emami Meybodi SM, Soleimani N, Yari A, et al. Circulatory long noncoding RNAs (circulatory-LNC-RNAs) as novel biomarkers and therapeutic targets in cardiovascular diseases: implications for cardiovascular diseases complications. Int J Biol Macromol 2023;225:1049-71.
44. Ao X, Ding W, Li X, et al. Non-coding RNAs regulating mitochondrial function in cardiovascular diseases. J Mol Med 2023;101:501-26.
45. Heshmatzad K, Naderi N, Maleki M, et al. Role of non-coding variants in cardiovascular disease. J Cell Mol Med 2023;27:1621-36.
46. Jiang X, Lei R. Extracellular lncRNAs secreted and absorbed by cardiomyocytes. J Cell Biochem 2023;124:785-96.
47. Zhu Y, Zhu L, Wang X, Jin H. RNA-based therapeutics: an overview and prospectus. Cell Death Dis 2022;13:644.
48. Gareri C, Polimeni A, Giordano S, Tammè L, Curcio A, Indolfi C. Antisense oligonucleotides and small interfering RNA for the treatment of dyslipidemias. J Clin Med 2022;11:3884.
49. Yeang C, Karwatowska-Prokopczuk E, Su F, et al. Effect of pelacarsen on lipoprotein(a) cholesterol and corrected low-density lipoprotein cholesterol. J Am Coll Cardiol 2022;79:1035-46.
50. Kim Y, Zharkinbekov Z, Sarsenova M, Yeltay G, Saparov A. Recent advances in gene therapy for cardiac tissue regeneration. Int J Mol Sci 2021;22:9206.
51. Brown EE, Murray B. A Practical guide to genetic testing in inherited heart disease. Card Electrophysiol Clin 2023;15:241-7.
52. Bowen JM, Hernandez M, Johnson DS, et al. Diagnosis and management of vascular Ehlers-Danlos syndrome: experience of the UK national diagnostic service, Sheffield. Eur J Hum Genet 2023;31:749-60.
53. Giuliani L, Di Toro A, Urtis M, et al. Prevalence and complications of aberrant subclavian artery in patients with heritable and nonheritable arteriopathies. J Am Coll Cardiol 2023;81:979-91.
54. Specterman MJ, Behr ER. Cardiogenetics: the role of genetic testing for inherited arrhythmia syndromes and sudden death. Heart 2023;109:434-41.
55. Asad ZUA, Krishan S, Roman D, Yousaf AF, Stavrakis S. Same gene, different story (a case report of congenital long QT syndrome subtype 8 with a novel mutation). Am J Cardiol 2023;200:13-7.
56. Auricchio A, Demarchi A, Özkartal T, et al. Role of genetic testing in young patients with idiopathic atrioventricular conduction disease. Europace 2023;25:643-50.
57. Lalaguna L, Ramos-hernández L, Priori SG, Lara-pezzi E. Genome editing and inherited cardiac arrhythmias. In: Xiao J, editor. Genome editing in cardiovascular and metabolic diseases. Singapore: Springer Nature; 2023. pp. 115-27.
58. Lloyd-Jones DM, Wang TJ, Leip EP, et al. Lifetime risk for development of atrial fibrillation: the Framingham heart study. Circulation 2004;110:1042-6.
59. Sagris M, Vardas EP, Theofilis P, Antonopoulos AS, Oikonomou E, Tousoulis D. Atrial fibrillation: pathogenesis, predisposing factors, and genetics. Int J Mol Sci 2021;23:6.
60. Kontorovich AR. Approaches to genetic screening in cardiomyopathies: practical guidance for clinicians. JACC Heart Fail 2023;11:133-42.
61. Brodehl A, Gerull B. Genetic insights into primary restrictive cardiomyopathy. J Clin Med 2022;11:2094.
62. Mansoori GA, Mahmeed WA, Wani S, et al. Introducing and implementing genetic assessment in cardio-obstetrics clinical practice: clinical and genetic workup of patients with cardiomyopathy. Int J Mol Sci 2023;24:9119.
63. Koslow M, Mondaca-Ruff D, Xu X. Transcriptome studies of inherited dilated cardiomyopathies. Mamm Genome 2023;34:312-22.
64. Ehrlich L, Prakash SK. Copy-number variation in congenital heart disease. Curr Opin Genet Dev 2022;77:101986.
65. Dotzler SM, Kim CSJ, Gendron WAC, et al. Suppression-replacement KCNQ1 gene therapy for type 1 long QT syndrome. Circulation 2021;143:1411-25.
66. Yamamoto Y, Makiyama T, Harita T, et al. Allele-specific ablation rescues electrophysiological abnormalities in a human iPS cell model of long-QT syndrome with a CALM2 mutation. Hum Mol Genet 2017;26:1670-7.
67. Vermersch E, Jouve C, Hulot JS. CRISPR/Cas9 gene-editing strategies in cardiovascular cells. Cardiovasc Res 2020;116:894-907.
68. Liu N, Olson EN. CRISPR modeling and correction of cardiovascular disease. Circ Res 2022;130:1827-50.
69. Kohaut E, Ader F, Rooryck C, et al. Morphological and genetic causes of fetal cardiomyopathies. Clin Genet 2023;104:63-72.
70. Lawley CM, Kaski JP. Clinical and genetic screening for hypertrophic cardiomyopathy in paediatric relatives: changing paradigms in clinical practice. J Clin Med 2023;12:2788.
71. Sheppard MN, van der Wal AC, Banner J, et al. Association for European Cardiovascular Pathology (AECVP). Genetically determined cardiomyopathies at autopsy: the pivotal role of the pathologist in establishing the diagnosis and guiding family screening. Virchows Arch 2023;482:653-69.
72. Robles-Mezcua A, Ruíz-Salas A, Medina-Palomo C, et al. The novel variant NP_00454563.2 (p.Glu259Glyfs*77) in gene PKP2 associated with arrhythmogenic cardiomyopathy in 8 families from Malaga, Spain. Genes 2023;14:1468.
73. Christensen KD, McMahon PM, Galbraith LN, et al. Benefits, harms, and costs of newborn genetic screening for hypertrophic cardiomyopathy: estimates from the PreEMPT model. Genet Med 2023;25:100797.
74. Burns C, Yeates L, Sweeting J, Semsarian C, Ingles J. Evaluating a communication aid for return of genetic results in families with hypertrophic cardiomyopathy: a randomized controlled trial. J Genet Couns 2023;32:425-34.
75. Chang CS, Hong SY, Kim SY, et al. Prevalence of associated extracardiac anomalies in prenatally diagnosed congenital heart diseases. PLoS One 2021;16:e0248894.
76. Formigari R, Michielon G, Digilio MC, et al. Genetic syndromes and congenital heart defects: how is surgical management affected? Eur J Cardiothorac Surg 2009;35:606-14.
77. Brunham LR, Hegele RA. What Is the Prevalence of familial hypercholesterolemia? Arterioscler Thromb Vasc Biol 2021;41:2629-31.
78. Knowles JW, O'Brien EC, Greendale K, et al. Reducing the burden of disease and death from familial hypercholesterolemia: a call to action. Am Heart J 2014;168:807-11.
79. Lagace TA. PCSK9 and LDLR degradation: regulatory mechanisms in circulation and in cells. Curr Opin Lipidol 2014;25:387-93.
80. Chambergo-Michilot D, Alur A, Kulkarni S, Agarwala A. Mipomersen in familial hypercholesterolemia: an update on health-related quality of life and patient-reported outcomes. Vasc Health Risk Manag 2022;18:73-80.
81. Wiegman A, Greber-Platzer S, Ali S, et al. Evinacumab for pediatric patients with homozygous familial hypercholesterolemia. Circulation 2024;149:343-53.
82. Adam RC, Mintah IJ, Alexa-Braun CA, et al. Angiopoietin-like protein 3 governs LDL-cholesterol levels through endothelial lipase-dependent VLDL clearance. J Lipid Res 2020;61:1271-86.
83. Karwatowska-Prokopczuk E, Lesogor A, Yan JH, et al. Efficacy and safety of pelacarsen in lowering Lp(a) in healthy Japanese subjects. J Clin Lipidol 2023;17:181-8.
84. Koren MJ, Moriarty PM, Baum SJ, et al. Preclinical development and phase 1 trial of a novel siRNA targeting lipoprotein(a). Nat Med 2022;28:96-103.
85. Kalia I, Shope R, Reilly M, Schwartz L. Addressing the underdiagnosis of familial hypercholesterolemia: a mixed methods study exploring the knowledge and practice behaviors of cardiology healthcare providers. J Clin Transl Sci 2023;7:e92.
86. Shuldiner AR, O'Connell JR, Bliden KP, et al. Association of cytochrome P450 2C19 genotype with the antiplatelet effect and clinical efficacy of clopidogrel therapy. JAMA 2009;302:849-57.
87. Gurbel PA, Tantry US, Shuldiner AR, Kereiakes DJ. Genotyping: one piece of the puzzle to personalize antiplatelet therapy. J Am Coll Cardiol 2010;56:112-6.
88. Tantry US, Bliden KP, Wei C, et al. First analysis of the relation between CYP2C19 genotype and pharmacodynamics in patients treated with ticagrelor versus clopidogrel: the ONSET/OFFSET and RESPOND genotype studies. Circ Cardiovasc Genet 2010;3:556-66.
89. Mega JL, Simon T, Collet JP, et al. Reduced-function CYP2C19 genotype and risk of adverse clinical outcomes among patients treated with clopidogrel predominantly for PCI: a meta-analysis. JAMA 2010;304:1821-30.
90. Gurbel PA, Bliden KP, Antonino MJ, et al. The effect of elinogrel on high platelet reactivity during dual antiplatelet therapy and the relation to CYP2C19*2 genotype: first experience in patients. J Thromb Haemost 2010;8:43-53.
91. Gurbel PA, Shuldiner AR, Bliden KP, Ryan K, Pakyz RE, Tantry US. The relation between CYP2C19 genotype and phenotype in stented patients on maintenance dual antiplatelet therapy. Am Heart J 2011;161:598-604.
92. Jeong YH, Tantry US, Kim IS, et al. Effect of CYP2C19*2 and *3 loss-of-function alleles on platelet reactivity and adverse clinical events in East Asian acute myocardial infarction survivors treated with clopidogrel and aspirin. Circ Cardiovasc Interv 2011;4:585-94.
93. Price MJ, Tantry US, Gurbel PA. The influence of CYP2C19 polymorphisms on the pharmacokinetics, pharmacodynamics, and clinical effectiveness of P2Y12 inhibitors. Rev Cardiovasc Med 2011;12:1-12.
94. Gurbel PA, Jeong YH, Tantry US. Personalized antiplatelet therapy: state of the art. JRSM Cardiovasc Dis 2012;1:1-10.
95. Gurbel PA, Tantry US. Do platelet function testing and genotyping improve outcome in patients treated with antithrombotic agents? Circulation 2012;125:1276-87.
96. Tantry US, Jeong YH, Navarese EP, Kubica J, Gurbel PA. Influence of genetic polymorphisms on platelet function, response to antiplatelet drugs and clinical outcomes in patients with coronary artery disease. Expert Rev Cardiovasc Ther 2013;11:447-62.
97. Jeong YH, Abadilla KA, Tantry US, et al. Influence of CYP2C19*2 and *3 loss-of-function alleles on the pharmacodynamic effects of standard- and high-dose clopidogrel in East Asians undergoing percutaneous coronary intervention: the results of the ACCEL-DOUBLE-2N3 study. J Thromb Haemost 2013;11:1194-7.
98. Erlinge D, James S, Duvvuru S, et al. Clopidogrel metaboliser status based on point-of-care CYP2C19 genetic testing in patients with coronary artery disease. Thromb Haemost 2014;111:943-50.
99. Gurbel PA, Bergmeijer TO, Tantry US, et al. The effect of CYP2C19 gene polymorphisms on the pharmacokinetics and pharmacodynamics of prasugrel 5-mg, prasugrel 10-mg and clopidogrel 75-mg in patients with coronary artery disease. Thromb Haemost 2014;112:589-97.
100. Jeong YH, Bliden KP, Shuldiner AR, Tantry US, Gurbel PA. Thrombin-induced platelet-fibrin clot strength: relation to high on-clopidogrel platelet reactivity, genotype, and post-percutaneous coronary intervention outcomes. Thromb Haemost 2014;111:713-24.
101. Doll JA, Neely ML, Roe MT, et al. TRILOGY ACS Investigators. Impact of CYP2C19 metabolizer status on patients with ACS treated with prasugrel versus clopidogrel. J Am Coll Cardiol 2016;67:936-47.
102. Winter MP, Grove EL, De Caterina R, et al. Advocating cardiovascular precision medicine with P2Y12 receptor inhibitors. Eur Heart J Cardiovasc Pharmacother 2017;3:221-34.
103. Verma SS, Bergmeijer TO, Gong L, et al. ICPC Investigators. Genomewide association study of platelet reactivity and cardiovascular response in patients treated with clopidogrel: a study by the international clopidogrel pharmacogenomics consortium. Clin Pharmacol Ther 2020;108:1067-77.
104. Lewis JP, Backman JD, Reny JL, et al. ICPC Investigators. Pharmacogenomic polygenic response score predicts ischaemic events and cardiovascular mortality in clopidogrel-treated patients. Eur Heart J Cardiovasc Pharmacother 2020;6:203-10.
105. Shawky A, Sabit H, Nazih M, Baraka K, El-Zawahry M. CYP2C19 polymorphism in ischemic heart disease patients taking clopidogrel after percutaneous coronary intervention in Egypt. J Epidemiol Glob Health 2023;13:374-83.
106. Mehta MP, Gajjar ND, Patel RJ, Joshi LP, Shah GB. Prevalence of CYP2C9 and CYP2C19 variants and the impact on clopidogrel efficacy in patients having CYPC19*2 variant. Indian J Pharmacol 2023;55:27-33.
107. Thomas CD, Williams AK, Lee CR, Cavallari LH. Pharmacogenetics of P2Y(12) receptor inhibitors. Pharmacotherapy 2023;43:158-75.
108. Berg JM, van den Broek WWA. Another step toward CYP2C19 genotype-guided therapy in treatment with dual antiplatelet therapy. JACC Cardiovasc Interv 2023;16:826-8.
109. Levens AD, den Haan MC, Jukema JW, et al. Feasibility of community pharmacist-initiated and point-of-care CYP2C19 genotype-guided de-escalation of oral P2Y12 inhibitors. Genes 2023;14:578.
110. Dong OM, Friede KA, Chanfreau-Coffinier C, Voora D. Cost-effectiveness of CYP2C19-guided P2Y12 inhibitors in Veterans undergoing percutaneous coronary intervention for acute coronary syndromes. Eur Heart J Qual Care Clin Outcomes 2023;9:249-57.
111. Koufaki MI, Fragoulakis V, Díaz-Villamarín X, et al. Economic evaluation of pharmacogenomic-guided antiplatelet treatment in Spanish patients suffering from acute coronary syndrome participating in the U-PGx PREPARE study. Hum Genomics 2023;17:51.
112. Silva GFD, Lopes BM, Moser V, Ferreira LE. Impact of pharmacogenetics on aspirin resistance: a systematic review. Arq Neuropsiquiatr 2023;81:62-73.
113. Herrera-Galeano JE, Becker DM, Wilson AF, et al. A novel variant in the platelet endothelial aggregation receptor-1 gene is associated with increased platelet aggregability. Arterioscler Thromb Vasc Biol 2008;28:1484-90.
114. Lewis JP, Riaz M, Xie S, et al. Genetic variation in PEAR1, cardiovascular outcomes and effects of aspirin in a healthy elderly population. Clin Pharmacol Ther 2020;108:1289-98.
115. Friede KA, Myers RA, Gales J, et al. An antiplatelet response gene expression signature is associated with bleeding. Cardiovasc Res 2023;119:551-60.
116. Tidbury N, Preston J, Lip GYH. Lessons learned from the influence of CYP2C9 genotype on warfarin dosing. Expert Opin Drug Metab Toxicol 2023;19:185-8.
117. Liu TY, Hsu HY, You YS, et al. Efficacy of warfarin therapy guided by pharmacogenetics: a real-world investigation among han taiwanese. Clin Ther 2023;45:662-70.
118. Šimičević L, Slišković AM, Kirhmajer MV, et al. Risk factors for rivaroxaban-related bleeding events-possible role of pharmacogenetics: case series. Pharmacy 2023;11:29.
119. Thompson LE, Davis BH, Narayan R, Goff B, Brown TM, Limdi NA. Personalizing direct oral anticoagulant therapy for a diverse population: role of race, kidney function, drug interactions, and pharmacogenetics. Clin Pharmacol Ther 2023;113:585-99.
120. Massmann A, Van Heukelom J, Green RC, et al. SLCO1B1 gene-based clinical decision support reduces statin-associated muscle symptoms risk with simvastatin. Pharmacogenomics 2023;24:399-409.
121. Shatnawi A, Kamran Z, Al-Share Q. Pharmacogenomics of lipid-lowering agents: the impact on efficacy and safety. Per Med 2023;20:65-86.
122. AlAzzeh O, Roman YM. The frequency of rs2231142 in ABCG2 among Native Hawaiian and Pacific Islander subgroups: implications for personalized rosuvastatin dosing. Pharmacogenomics 2023;24:173-82.
123. Alrajeh K, Roman YM. The frequency of rs2231142 in ABCG2 among Asian subgroups: implications for personalized rosuvastatin dosing. Pharmacogenomics 2023;24:15-26.
124. Tornio A, Bigossi M, Siddiqui MK, et al. The cholesterol-lowering effect of statins is modified by LILRB5 intolerance genotype: Results from a recruit-by-genotype clinical trial. Front Pharmacol 2023;14:1090010.
125. Valverde-Hernández JC, Flores-Cruz A, Chavarría-Soley G, Silva de la Fuente S, Campos-Sánchez R. Frequencies of variants in genes associated with dyslipidemias identified in Costa Rican genomes. Front Genet 2023;14:1114774.
126. Dawed AY, Mari A, Brown A, et al. DIRECT consortium. Pharmacogenomics of GLP-1 receptor agonists: a genome-wide analysis of observational data and large randomised controlled trials. Lancet Diabetes Endo 2023;11:33-41.
127. Sarhan N, Essam Abou Warda A, Alsahali S, Alanazi AS. Impact of vitamin D supplementation on the clinical outcomes and epigenetic markers in patients with acute coronary syndrome. Pharmaceuticals 2023;16:262.
128. Oluwole OG, Henry M. Genomic medicine in Africa: a need for molecular genetics and pharmacogenomics experts. Curr Med Res Opin 2023;39:141-7.
129. Oni-Orisan A, Tuteja S, Hoffecker G, et al. Pharmacogenomics Global Research Network (PGRN) Publications Committee. An introductory tutorial on cardiovascular pharmacogenetics for healthcare providers. Clin Pharmacol Ther 2023;114:275-87.
130. Saleh A, Al-Abcha A, Pereira N. Pharmacogenetics of cardiovascular drugs. Curr Opin Cardiol 2023;38:207-14.
131. Nogueiras-Álvarez R. Pharmacogenomics in clinical trials: an overview. Front Pharmacol 2023;14:1247088.
132. Tardif JC, Pfeffer MA, Dubé MP, Investigators dG. Pharmacogenetics-guided dalcetrapib therapy after an acute coronary syndrome: the dal-GenE trial. Eur Heart J 2022;43:5062-3.
133. Ommen SR, Mital S, Burke MA, et al. 2020 AHA/ACC guideline for the diagnosis and treatment of patients with hypertrophic cardiomyopathy: a report of the American college of cardiology/American heart association joint committee on clinical practice guidelines. Circulation 2020;142:e558-631.
134. Musunuru K, Hershberger RE, Day SM, et al. Genetic testing for inherited cardiovascular diseases: a scientific statement from the American heart association. Circ Genom Precis Med 2020;13:e000067.
135. Landstrom AP, Kim JJ, Gelb BD, et al. Genetic testing for heritable cardiovascular diseases in pediatric patients: a scientific statement from the American heart association. Circ Genom Precis Med 2021;14:e000086.
136. Isselbacher EM, Preventza O, Hamilton Black J 3rd, et al. 2022 ACC/AHA guideline for the diagnosis and management of aortic disease: a report of the American heart association/American college of cardiology joint committee on clinical practice guidelines. Circulation 2022;146:e334-482.
137. Landstrom AP, Chahal AA, Ackerman MJ, et al. Interpreting incidentally identified variants in genes associated with heritable cardiovascular disease: a scientific statement from the American heart association. Circ Genom Precis Med 2023;16:e000092.
138. Wilde AAM, Semsarian C, Márquez MF, et al. European Heart Rhythm Association (EHRA)/Heart Rhythm Society (HRS)/Asia Pacific Heart Rhythm Society (APHRS)/Latin American Heart Rhythm Society (LAHRS) expert consensus statement on the state of genetic testing for cardiac diseases. Europace 2022;24:1307-67.
139. Genetic testing for cardiac disease. Available from: https://www.uhcprovider.com/content/dam/provider/docs/public/policies/oxford/gene-expression-tests-cardiac-conditions-ohp.pdf [Last accessed on 12 Apr 2024].
140. Genetic testing for cardiovascular disease. Available from: https://www.cms.gov/medicare-coverage-database/view/lcd.aspx?lcdId=39084&ver=5 [Last accessed on 12 Apr 2024].
141. GeneDx. Genetic testing for cardiovascular disease. Available from: https://www.cms.gov/medicare-coverage-database/view/lcd.aspx?lcdId=39084&ver=5#:~:text=However%2C%20Medicare%20does%20not%20cover,diagnostic%20result%20in%20most%20circumstances [Last accessed on 15 Apr 2024].
142. Abdullah-Koolmees H, van Keulen AM, Nijenhuis M, Deneer VHM. Pharmacogenetics guidelines: overview and comparison of the DPWG, CPIC, CPNDS, and RNPGx guidelines. Front Pharmacol 2020;11:595219.
143. Tayeh MK, Gaedigk A, Goetz MP, et al. ACMG Laboratory Quality Assurance Committee. Electronic address: documents@acmg.net. Clinical pharmacogenomic testing and reporting: a technical standard of the American College of Medical Genetics and Genomics (ACMG). Genet Med 2022;24:759-68.
144. Table of pharmacogenetic associations. Available from: https://www.fda.gov/medical-devices/precision-medicine/table-pharmacogenetic-associations [Last accessed on 12 Apr 2024].
145. Johnson JA, Caudle KE, Gong L, et al. Clinical pharmacogenetics implementation consortium (CPIC) guideline for pharmacogenetics-guided warfarin dosing: 2017 Update. Clin Pharmacol Ther 2017;102:397-404.
146. Sibbing D, Aradi D, Alexopoulos D, et al. Updated expert consensus statement on platelet function and genetic testing for Guiding P2Y12 receptor inhibitor treatment in percutaneous coronary intervention. JACC Cardiovasc Interv 2019;12:1521-37.
147. Lee CR, Luzum JA, Sangkuhl K, et al. Clinical pharmacogenetics implementation consortium guideline for CYP2C19 genotype and clopidogrel therapy: 2022 update. Clin Pharmacol Ther 2022;112:959-67.
148. Cooper-DeHoff RM, Niemi M, Ramsey LB, et al. The clinical pharmacogenetics implementation consortium guideline for SLCO1B1, ABCG2, and CYP2C9 genotypes and statin-associated musculoskeletal symptoms. Clin Pharmacol Ther 2022;111:1007-21.
149. MolDX: pharmacogenomics testing. Available from: https://www.cms.gov/medicare-coverage-database/view/lcd.aspx?LCDId=38294&ver=16 [Last accessed on 12 Apr 2024].
150. Pritchard D, Patel JN, Stephens LE, McLeod HL. Comparison of FDA table of pharmacogenetic associations and clinical pharmacogenetics implementation consortium guidelines. Am J Health Syst Pharm 2022;79:993-1005.
151. Dellefave-Castillo LM, Cirino AL, Callis TE, et al. Assessment of the diagnostic yield of combined cardiomyopathy and arrhythmia genetic testing. JAMA Cardiol 2022;7:966-74.
152. Mighton C, Shickh S, Uleryk E, Pechlivanoglou P, Bombard Y. Clinical and psychological outcomes of receiving a variant of uncertain significance from multigene panel testing or genomic sequencing: a systematic review and meta-analysis. Genet Med 2021;23:22-33.
153. Richards S, Aziz N, Bale S, et al. ACMG Laboratory Quality Assurance Committee. Standards and guidelines for the interpretation of sequence variants: a joint consensus recommendation of the American College of Medical Genetics and Genomics and the Association for Molecular Pathology. Genet Med 2015;17:405-24.
154. Reuter C, Chun N, Pariani M, Hanson-Kahn A. Understanding variants of uncertain significance in the era of multigene panels: through the eyes of the patient. J Genet Couns 2019;28:878-86.
155. Wong EK, Bartels K, Hathaway J, et al. Perceptions of genetic variant reclassification in patients with inherited cardiac disease. Eur J Hum Genet 2019;27:1134-42.
156. Gordon AS, Zouk H, Venner E, et al. Clinical Annotation Working Group. Frequency of genomic secondary findings among 21,915 eMERGE network participants. Genet Med 2020;22:1470-7.
157. McKnight D, Morales A, Hatchell KE, et al. ELEVIATE Consortium. Genetic testing to inform epilepsy treatment management from an international study of clinical practice. JAMA Neurol 2022;79:1267-76.
158. Lemke AA, Amendola LM, Thompson J, et al. Patient-reported outcomes and experiences with population genetic testing offered through a primary care network. Genet Test Mol Biomarkers 2021;25:152-60.
159. Sanderson SC, Linderman MD, Suckiel SA, et al. Psychological and behavioural impact of returning personal results from whole-genome sequencing: the HealthSeq project. Eur J Hum Genet 2017;25:280-92.
160. Abdallaoui OEA, Tornyos D, Lukács R, Szabó D, Komócsi A. Individualized or uniform de-escalation strategies for antiplatelet therapy in acute coronary syndrome: a review of clinical trials with platelet function testing and genetic testing-based protocols. Int J Mol Sci 2023;24:9071.
161. Nomura A, Okada H, Nohara A, Kawashiri MA, Takamura M, Tada H. Impact of providing genetics-based future cardiovascular risk on LDL-C in patients with familial hypercholesterolemia. J Clin Lipidol 2023;17:622-32.
162. Verdonschot JAJ, Hazebroek MR, Krapels IPC, et al. Implications of genetic testing in dilated cardiomyopathy. Circ Genom Precis Med 2020;13:476-87.
163. Bradford WH, Zhang J, Gutierrez-lara EJ, et al. Plakophilin 2 gene therapy prevents and rescues arrhythmogenic right ventricular cardiomyopathy in a mouse model harboring patient genetics. Nat Cardiovasc Res 2023;2:1246-61.
164. van Opbergen CJM, Narayanan B, Sacramento CB, et al. AAV-mediated delivery of Plakophilin-2a arrests progression of arrhythmogenic right ventricular cardiomyopathy in murine hearts: preclinical evidence supporting gene therapy in humans. Circ Genom Precis Med 2024;17:e004305.
165. Morales A, Goehringer J, Sanoudou D. Evolving cardiovascular genetic counseling needs in the era of precision medicine. Front Cardiovasc Med 2023;10:1161029.
166. Rickman AF, Fitzgerald-Butt SM, Spoonamore KG, Ware SM, Helm BM. A descriptive investigation of clinical practice models used by cardiovascular genetic counselors in North America. J Genet Couns 2023;32:362-75.
167. Christian S, Dzwiniel T. Principles of genetic counseling in inherited heart conditions. Card Electrophysiol Clin 2023;15:229-39.
168. Girolami F, Gozzini A, Pálinkás ED, et al. Genetic testing and counselling in hypertrophic cardiomyopathy: frequently asked questions. J Clin Med 2023;12:2489.
169. Tromp TR, Reijman MD, Wiegman A, et al. Counseling couples at risk of having a child with homozygous familial hypercholesterolemia - clinical experience and recommendations. J Clin Lipidol 2023;17:291-6.
170. O'Sullivan JW, Raghavan S, Marquez-Luna C, et al. Polygenic risk scores for cardiovascular disease: a scientific statement from the American Heart Association. Circulation 2022;146:e93-118.
171. Abraham G, Havulinna AS, Bhalala OG, et al. Genomic prediction of coronary heart disease. Eur Heart J 2016;37:3267-78.
172. Natarajan P, Young R, Stitziel NO, et al. Polygenic risk score identifies subgroup with higher burden of atherosclerosis and greater relative benefit from statin therapy in the primary prevention setting. Circulation 2017;135:2091-101.
173. Inouye M, Abraham G, Nelson CP, et al. UK Biobank CardioMetabolic Consortium CHD Working Group. Genomic risk prediction of coronary artery disease in 480,000 adults: implications for primary prevention. J Am Coll Cardiol 2018;72:1883-93.
174. O'Sullivan JW, Ashley EA, Elliott PM. Polygenic risk scores for the prediction of cardiometabolic disease. Eur Heart J 2023;44:89-99.
175. Weindling P. The origins of informed consent: the international scientific commission on medical war crimes, and the Nuremberg code. Bull Hist Med 2001;75:37-71.
176. World Medical Association Inc. Declaration of Helsinki. Ethical principles for medical research involving human subjects. J Indian Med Assoc 2009;107:403-5.
177. Nurmi SM, Halkoaho A, Moilanen J, Remes AM, Solje E. The ethical implications of genetic testing in neurodegenerative diseases: a systematic review. Scand J Caring Sci 2021;35:1057-74.
178. Ascencio-Carbajal T, Saruwatari-Zavala G, Navarro-Garcia F, Frixione E. Genetic/genomic testing: defining the parameters for ethical, legal and social implications (ELSI). BMC Med Ethics 2021;22:156.
179. Panacer KS. Ethical issues associated with direct-to-consumer genetic testing. Cureus 2023;15:e39918.
180. Beig Goharrizi MA, Ghodsi S, Memarjafari MR. Implications of CRISPR-Cas9 genome editing methods in atherosclerotic cardiovascular diseases. Curr Probl Cardiol 2023;48:101603.
181. Li ZH, Wang J, Xu JP, Wang J, Yang X. Recent advances in CRISPR-based genome editing technology and its applications in cardiovascular research. Mil Med Res 2023;10:12.
182. Morris ZS, Wooding S, Grant J. The answer is 17 years, what is the question: understanding time lags in translational research. J R Soc Med 2011;104:510-20.
183. Mathur A, Mohan V, Ameta D, Gaurav B, Haranahalli P. Aortic aneurysm. J Transl Int Med 2016;4:35-41.
185. Krahn AD, Laksman Z, Sy RW, et al. Congenital long QT syndrome. JACC Clin Electrophysiol 2022;8:687-706.
186. Schwartz PJ, Crotti L, Insolia R. Long-QT syndrome: from genetics to management. Circ Arrhythm Electrophysiol 2012;5:868-77.
187. Anttonen O, Junttila MJ, Rissanen H, Reunanen A, Viitasalo M, Huikuri HV. Prevalence and prognostic significance of short QT interval in a middle-aged Finnish population. Circulation 2007;116:714-20.
188. Guerrier K, Kwiatkowski D, Czosek RJ, Spar DS, Anderson JB, Knilans TK. Short QT interval prevalence and clinical outcomes in a pediatric population. Circ Arrhythm Electrophysiol 2015;8:1460-4.
189. Pérez-Riera AR, Barbosa-Barros R, de Rezende Barbosa MPC, Daminello-Raimundo R, de Lucca AA Jr, de Abreu LC. Catecholaminergic polymorphic ventricular tachycardia, an update. Ann Noninvasive Electrocardiol 2018;23:e12512.
190. Krahn AD, Wilde AAM, Calkins H, et al. Arrhythmogenic right ventricular cardiomyopathy. JACC Clin Electrophysiol 2022;8:533-53.
191. Krahn AD, Behr ER, Hamilton R, Probst V, Laksman Z, Han HC. Brugada syndrome. JACC Clin Electrophysiol 2022;8:386-405.
192. Maron BJ, Desai MY, Nishimura RA, et al. Diagnosis and evaluation of hypertrophic cardiomyopathy: JACC state-of-the-art review. J Am Coll Cardiol 2022;79:372-89.
193. Heymans S, Lakdawala NK, Tschöpe C, Klingel K. Dilated cardiomyopathy: causes, mechanisms, and current and future treatment approaches. Lancet 2023;402:998-1011.
194. Fatkin D, Calkins H, Elliott P, James CA, Peters S, Kovacic JC. Contemporary and future approaches to precision medicine in inherited cardiomyopathies: JACC focus seminar 3/5. J Am Coll Cardiol 2021;77:2551-72.
195. van der Linde D, Konings EE, Slager MA, et al. Birth prevalence of congenital heart disease worldwide: a systematic review and meta-analysis. J Am Coll Cardiol 2011;58:2241-7.
196. Beheshti SO, Madsen CM, Varbo A, Nordestgaard BG. Worldwide prevalence of familial hypercholesterolemia: meta-analyses of 11 million subjects. J Am Coll Cardiol 2020;75:2553-66.
197. Tada H, Nohara A, Inazu A, Sakuma N, Mabuchi H, Kawashiri MA. Sitosterolemia, hypercholesterolemia, and coronary artery disease. J Atheroscler Thromb 2018;25:783-9.
198. Tada H, Nomura A, Ogura M, et al. Diagnosis and management of sitosterolemia 2021. J Atheroscler Thromb 2021;28:791-801.
199. Ruopp NF, Cockrill BA. Diagnosis and treatment of pulmonary arterial hypertension: a review. JAMA 2022;327:1379-91.
Cite This Article
Export citation file: BibTeX | RIS
OAE Style
Bliden K, Singh S, Shanoada R, Kalia I, Tantry U, Zimmerman A, Babu AD, Raghavakurup L, Stude T, Sidorski D, Gurbel P. Genetics in the diagnosis and treatment of cardiovascular diseases. J Transl Genet Genom 2024;8:186-206. http://dx.doi.org/10.20517/jtgg.2023.59
AMA Style
Bliden K, Singh S, Shanoada R, Kalia I, Tantry U, Zimmerman A, Babu AD, Raghavakurup L, Stude T, Sidorski D, Gurbel P. Genetics in the diagnosis and treatment of cardiovascular diseases. Journal of Translational Genetics and Genomics. 2024; 8(2): 186-206. http://dx.doi.org/10.20517/jtgg.2023.59
Chicago/Turabian Style
Bliden, Kevin, Sahib Singh, Roni Shanoada, Isha Kalia, Udaya Tantry, Alyssa Zimmerman, Aravind Dilli Babu, Lekshminarayan Raghavakurup, Taylor Stude, Damian Sidorski, Paul Gurbel. 2024. "Genetics in the diagnosis and treatment of cardiovascular diseases" Journal of Translational Genetics and Genomics. 8, no.2: 186-206. http://dx.doi.org/10.20517/jtgg.2023.59
ACS Style
Bliden, K.; Singh S.; Shanoada R.; Kalia I.; Tantry U.; Zimmerman A.; Babu AD.; Raghavakurup L.; Stude T.; Sidorski D.; Gurbel P. Genetics in the diagnosis and treatment of cardiovascular diseases. J. Transl. Genet. Genom. 2024, 8, 186-206. http://dx.doi.org/10.20517/jtgg.2023.59
About This Article
Special Issue
Copyright
Data & Comments
Data
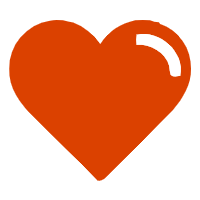

Comments
Comments must be written in English. Spam, offensive content, impersonation, and private information will not be permitted. If any comment is reported and identified as inappropriate content by OAE staff, the comment will be removed without notice. If you have any queries or need any help, please contact us at support@oaepublish.com.